Abstract
Graphene-containing 13-93 bioactive glass and poly(ε-caprolactone)-based bilayer, electrically conductive scaffolds were prepared for osteochondral tissue repair. Biological response of osteoblastic MC3T3-E1 and chondrogenic ATDC5 cells to the composite scaffolds was assessed under mono-culture and co-culture conditions. Cytotoxicity was investigated using MTT assay, cartilage matrix production was evaluated by Alcian blue staining, and mineralization of both types of cells in the different culture systems was observed by Alizarin red S staining. Results showed that osteoblastic and chondrogenic cells utilized in the study did not show toxic response to the prepared scaffolds under mono-culture conditions and higher cell viability rates were obtained in co-culture conditions. Larger mineralized areas were determined under co-culture conditions and calcium deposition amount significantly increased compared with that in control group samples after 21 days. Additionally, the amount of glycosaminoglycans synthesized in co-culture was higher compared to mono-culture conditions. Electric stimulation applied under mono-culture conditions suppressed the viability of MC3T3-E1 cells whereas it enhanced the viability rates of ATDC5 cells. The study suggests that the designed bilayered osteochondral constructs have the potential for osteochondral defect repair.








Similar content being viewed by others
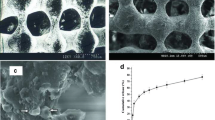
References
Nukavarapu, S. P., & Dorcemus, D. L. (2013). Osteochondral tissue engineering: current strategies and challenges. Biotechnology Advances, 31(5), 706–721.
Zhang, L., Hu, J., & Athanasiou, K. A. (2009). The role of tissue engineering in articular cartilage repair and regeneration. Critical Reviews in Biomedical Engineering, 37(1–2), 1–57.
He, A., Liu, L., Luo, X., Liu, Y., Liu, Y., Liu, F., Wang, X., Zhang, Z., Zhang, W., Liu, W., Cao, Y., & Zhou, G. (2017). Repair of osteochondral defects with in vitro engineered cartilage based on autologous bone marrow stromal cells in a swine model. Scientific Reports, 7, 40489.
Naghizadeh, F., Solouk, A., & Khoulenjani, S. B. (2017). Osteochondral scaffolds based on electrospinning method: general review on new and emerging approaches. International Journal of Polymeric Materials and Polymeric Biomaterials, 1–12. https://doi.org/10.1080/00914037.2017.1393682.
Martin, I., Miot, S., Barbero, A., Jakob, M., & Wendt, D. (2007). Osteochondral tissue engineering. Journal of Biomechanics, 40(4), 750–765.
Mardones, R., Jofré, C. M., & Minguell, J. J. (2015). Cell therapy and tissue engineering approaches for cartilage repair and/or regeneration. International Journal of Stem Cells., 8(1), 48–53.
Gobbi, A., Kon, E., Berruto, M., Filardo, G., Delcogliano, M., & Boldrini, L. (2009). Patellofemoral full-thickness chondral defects treated with second-generation autologous chondrocyte implantation. American Journal of Sports Medicine, 37, 234–242.
Lee, P., Manoukian, O. S., Zhou, G., Wang, Y., Chang, W., Yua, X., & Kumbar, S. G. (2016). Osteochondral scaffold combined with aligned nanofibrous scaffolds for cartilage regeneration. RSC Advances, 6(76), 72246–72255.
Nooeaid, P., Salih, V., Beier, J. P., & Boccaccini, A. R. (2012). Osteochondral tissue engineering: scaffolds, stem cells and applications. Journal of Cellular and Molecular Medicine., 16(10), 2247–2270.
Zhu, Y., Song, K., Jiang, S., Chen, J., Tang, L., Li, S., Fan, J., Wang, Y., Zhao, J., & Liu, T. (2017). Numerical simulation of mass transfer and three-dimensional fabrication of tissue-engineered cartilages based on chitosan/gelatin hybrid hydrogel scaffold in a rotating bioreactor. Applied Biochemistry and Biotechnology, 181(1), 250–266.
Jeong, C. G., Zhang, H., & Hollister, S. J. (2012). Three-dimensional polycaprolactone scaffold conjugated bone morphogenetic protein-2 promotes cartilage regeneration from primary chondrocytes in vitro and in vivo without accelerated endochondral ossification. Journal of Biomedical Materials Research, Part A, 2012, 100A.
Cao, T., Ho, K.-H., & Teoh, S.-H. (2003). Scaffold design and in vitro study of osteochondral coculture in a three-dimensional porous polycaprolactone scaffold fabricated by fused deposition modeling. Tissue Engineering, 9(1), 23–34.
Oliveira, J. M., Rodrigues, M. T., Silva, S. S., Malafaya, P. B., Gomes, M. E., & Viegas, C. A. (2006). Novel hydroxyapatite/chitosan bilayered scaffold for osteochondral tissue-engineering applications: scaffold design and its performance when seeded with goat bone marrow stromal cells. Biomaterials, 27, 897–907.
Jeon, J. E., Vaquette, C., Klein, T. J., & Hutmacher, D. W. (2014). Perspectives in multiphasic osteochondral tissue engineering. The Anatomical Record, 297(1), 26–35.
Çakmak, S., Çakmak, A. S., Kaplan, D. L., & Gümüsderelioglu, M. (2016). A silk fibroin and peptide amphiphile-based co-culture model for osteochondral tissue engineering. Macromolecular Bioscience, 16(8), 1212–1226. https://doi.org/10.1002/mabi.201600013.
Chang, C. H., Lin, F. H., Lin, C. C., Chou, C. H., & Liu, H. C. (2004). Cartilage tissue engineering on the surface of a novel gelatincalcium-phosphate biphasic scaffold in a double-chamber bioreactor. Journal of Biomedical Materials Research, 71B(2), 313–321.
Schek, R. M., Taboas, J. M., Segvich, S. J., Hollister, S. J., & Krebsbach, P. H. (2004). Engineered osteochondral grafts using biphasic composite solid free-form fabricated scaffolds. Tissue Engineering, 10(9–10), 1376–1385.
Yunos, D. M., Ahmad, Z., Salih, V., & Boccaccini, A. R. (2013). Stratified scaffolds for osteochondral tissue engineering applications: electrospun PDLLA nanofibre coated Bioglassfi-derived foams. Journal of Biomaterials Applications, 27(5), 537–551.
Jayabalan, P., Tan, A. R., & Rahaman, M. N. (2011). Bioactive glass 13-93 as a subchondral substrate for tissue-engineered osteochondral constructs. Clinical Orthopaedics and Related Research, 469(10), 2754–2763.
Yao, Q., Nooeaid, P., Detsch, R., Roether, J. A., Dong, Y., Goudouri, O.-M., Schubert, D. W., & Boccaccini, A. R. (2014). Bioglass/chitosan–polycaprolactone bilayered composite scaffolds intended for osteochondral tissue engineering. Journal of Biomedical Materials Research, Part A, 102(12), 4510–4518.
Fu, Q., Rahaman, M. N., Fu, H., & Liu, X. (2010). Silicate, borosilicate, and borate bioactive glass scaffolds with controllable degradation rate for bone tissue engineering applications. I. Preparation and in vitro degradation. Journal of Biomedical Materials Research, Part A, 95((1), 164–171.
Riss, T.L., Moravec, R.A., Niles, A.L., Duellman, S., Benink, H.A., Worzella, T.J., Minor, L. (2004). Cell viability assays. In: Sittampalam GS, Coussens NP, Brimacombe K, editors. Bethesda (MD): Eli Lilly & Company and the National Center for Advancing Translational Sciences.
Izadifar, Z., Chen, X., & Kulyk, W. (2012). Strategic design and fabrication of engineered scaffolds for articular cartilage repair. Journal of Functional Biomaterials, 3(4), 799–838.
Beck, G. R. (2003). Inorganic phosphate as a signaling molecule in osteoblast differentiation. Journal of Cellular Biochemistry, 90, 234–243.
Yang, L., Tsang, K. Y., Tang, H. C., Chan, D., & Cheah, K. S. E. (2014). Hypertrophic chondrocytes can become osteoblasts and osteocytes in endochondral bone formation. Proceedings of the National Academy of Sciences of the United States of America, 111(33), 12097–12102.
Çakmak, A.S. (2014). Investigation of osteogenic differentiation of mesenchymal stem cells with scaffolds supported by biophysical and biochemical stimulants, Hacettepe University, PhD thesis.
Björnsson, S. (1998). Quantitation of proteoglycans as glycosaminoglycans in biological fluids using an Alcian blue dot blot analysis. Analytical Biochemistry, 256(2), 229–237.
Wuthier, R. E. (1993). Involvement of cellular metabolism of calcium and phosphate in calcification of avian growth plate cartilage. The Journal of Nutrition, 123(suppl_2), 301–309.
Loh, Q. L., & Choong, C. C. (2013). Three-dimensional scaffolds for tissue engineering applications: role of porosity and pore size. Tissue Engineering Part B, 19(6), 485–502.
Liu, H., Lee, Y. W., & Dean, M. F. (1998). Re-expression of differentiated proteoglycan phenotype by dedifferentiated human chondrocytes during culture in alginate beads. Biochimica et Biophysica Acta, 1425(3), 505–515.
Yuan, X., Arkonac, D. E., Chao, P. G., & Vunjak-Novakovic, G. (2014). Electrical stimulation enhances cell migration and integrative repair in the meniscus. Scientific Reports, 4, 3674.
Pilla, A., Fitzsimmons, R., Muehsam, D., Wu, J., Rohde, C., & Casper, D. (2011). Electromagnetic fields as first messenger in biological signaling: application to calmodulin-dependent signaling in tissue repair. Biochimica et Biophysica Acta, 1810(12), 1236–1245.
Bobrinetskiy, I. I., Seleznev, A. S., Morozov, R. A., Lopatina, O. A., Podchernyaeva, R. Y., & Suetina, I. A. (2012). Investigation of the effect of local electrical stimulation on cells cultured on conductive single-walled carbon nanotube/albumin films. Journal of Biomaterials and Nanobiotechnology, 3(03), 377–384.
Brighton, C. T., Wang, W., Seldes, R., Zhang, G., & Pollack, S. R. (2001). Signal transduction in electrically stimulated bone cells. The Journal of Bone and Joint Surgery, 83-A(10), 1514–1523.
Zhuang, H., Wang, W., Seldes, R. M., Tahernia, A. D., Fan, H., & Brighton, C. T. (1997). Electrical stimulation induces the level of TGF-beta1 mRNA in osteoblastic cells by a mechanism involving calcium/calmodulin pathway. Biochemical and Biophysical Research Communications, 237(2), 225–229.
Wang, Y., Cui, H., Wu, Z., Wu, N., Wang, Z., Chen, X., Wei, Y., & Zhang, P. (2016). Modulation of osteogenesis in MC3T3-E1 cells by different frequency electrical stimulation. PLoS One, 11(5), e0154924.
Meng, S. Y., Rouabhia, M., & Zhang, Z. (2011). Accelerated osteoblast mineralization on a conductive substrate by multiple electrical stimulation. Journal of Bone and Mineral Metabolism, 29(5), 535–544.
Vaca-González, J. J., Guevara, J. M., Vega, J. F., & Garzón-Alvarado, D. A. (2016). An in vitro chondrocyte electrical stimulation framework: a methodology to calculate electric fields and modulate proliferation, cell death and glycosaminoglycan synthesis. Cellular and Molecular Bioengineering, 9(1), 116–126.
Nakasuji, S., Morita, Y., Tanaka, K., Katayama, T., & Nakamachi, E. (2009). Effect of electric field stimulation on chondrocyte activity. The proceedings of the JSME Annual Meeting. https://doi.org/10.1299/jsmemecjo.2009.6.0_293.
Wang, W., Wang, Z., Zhang, G., Clark, C. C., & Brighton, C. T. (2004). Up-regulation of chondrocyte matrix genes and products by electric fields. Clinical Orthopaedics and Related Research, 427, 163–173.
Acknowledgments
Cell culture experiments were performed in Applied Science Research Center at Manisa Celal Bayar University.
Funding
The financial support for this research was provided by the Scientific and Technical Research Council of Turkey (TUBITAK), 1001 grant program for scientific and technological research projects; grant no: 114M519.
Author information
Authors and Affiliations
Corresponding author
Ethics declarations
Conflict of Interest
The authors declare that they have no conflict of interest.
Ethical Approval
This article does not contain any studies with human participants or animals.
Rights and permissions
About this article
Cite this article
Deliormanlı, A.M., Atmaca, H. Biological Response of Osteoblastic and Chondrogenic Cells to Graphene-Containing PCL/Bioactive Glass Bilayered Scaffolds for Osteochondral Tissue Engineering Applications. Appl Biochem Biotechnol 186, 972–989 (2018). https://doi.org/10.1007/s12010-018-2758-7
Received:
Accepted:
Published:
Issue Date:
DOI: https://doi.org/10.1007/s12010-018-2758-7