Abstract
Enhancing use efficiency of applied fertiliser increases farmers’ returns on fertiliser investment through reducing nutrient inputs and improving yields. We investigated on-farm how indigenous nutrient supply and management practices affected N, P, and K uptake, agronomic efficiency and recovery efficiency of fertiliser, and physiological efficiency of nutrients taken up, under irrigated lowland rice conditions in Uganda. Treatments included recommended agronomic practices (RAP) without fertilisation, farmers’ practice (FP), farmers’ selected intensification practice (FIP), and RAP with NPK fertilisation (RAP + NPK). Indigenous N, P, and K supply varied greatly among farmers’ fields. N, P, and K uptake were significantly higher under RAP + NPK than under RAP, FP, and FIP; however, physiological efficiency (PE; kg grain kg−1 nutrient uptake) was significantly lower under RAP + NPK by 19% (N), and 12% (P/K), due to a larger effect of NPK application on uptake than on yield, leading to higher tissue concentrations. Indigenous available N reduced apparent N recovery, and agronomic and physiological N efficiencies independent of treatment. Also, P and K PEs decreased with increasing indigenous supply. Delaying weeding beyond recommended time, in interaction with indigenous N supply, decreased agronomic N efficiency, but increased PE of N. Interaction between P rate and timing reduced its PE; K rate and weeding time interaction reduced its PE. The decrease in efficiencies at high indigenous supply and delayed weeding indicates a need for site-specific fertilisation strategies based on naturally available nutrient levels and proper weeding. Weeding and fertilisation timing directly affect nutrient use efficiency, and therefore, fertiliser use efficiency in rice production systems.
Similar content being viewed by others
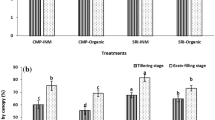
Avoid common mistakes on your manuscript.
Introduction
Rice (Oryza sativa) is one of the most important food crops in sub-Saharan Africa (SSA), the most rapidly growing food commodity, and the second largest source of caloric intake after maize (Bado et al. 2018; Tsujimoto et al. 2019). Rice is mostly grown by smallholder farmers, and yields are generally low. Yet, demand is projected to continue to rise due to high population growth rate, rapid urbanisation, and increasing consumer preference for rice (Balasubramanian et al. 2007; Tanaka et al. 2017; Tsujimoto et al. 2019). Because of the low yields, current local production does not meet the growing demand, and consumption is satisfied through imports. However, import dependence to meet the demand could worsen food insecurity and poverty (Tanaka et al. 2017). Moreover, improving rice production is not only important for improving country-level food security, but is also vital for enhancing household income, alleviating poverty, and promoting socio-economic growth of rice farmers (Seck et al. 2013; Tanaka et al. 2015).
Fertilisers are reported as a yield enhancing input (Awio et al. 2021; Bado et al. 2018; Kwesiga et al. 2019; Niang et al. 2017; Saito et al. 2019). While rice yields can be augmented through fertilisation, large variation in yield responses to fertiliser application has been observed among farmers’ fields within the same growing environment. For instance, in a participatory study under irrigated lowland condition on farmers’ fields in Uganda, grain yields ranged from 4.3 to 7.0 t ha−1 under researcher-supervised management practices including 100, 50 and 50 kg ha−1 N, P and K applications, respectively (Awio et al. 2022). Under farmers’ N rates ranging from 6.8 to 46 kg N ha−1, grain yield varied between 3.2 and 6.5 t ha−1. Likewise, Saito et al. (2019) reported grain yields between 0.8 and 2.3 t ha−1 on-farm with 160, 25 and 70 kg ha−1 N, P and K, respectively, at the same location in Uganda. In several other rice growing environments in SSA, this large yield variation among fields has been observed under the same growing conditions, when the same amounts of N, P and K were applied; with the highest variation of 0.1 to 7.2 t ha−1 grain yield with 110, 20 and 50 kg ha−1 N, P and K, respectively, observed in Benin under rainfed lowland condition (Saito et al. 2019). These differences in yield response to fertilisation among fields under similar growing conditions could reflect differences in soil indigenous nutrient supply (Haefele and Wopereis 2005; Kihara and Njoroge 2013; Vanlauwe et al. 2006). Indigenous nutrient supply is “the total amount of a particular nutrient that is available to crops from the soil during a crop** cycle”, as “estimated by measuring plant nutrient uptake in a nutrient omission plot” (Witt and Dobermann 2002). It can originate from incorporated crop residues, residual organic and inorganic nutrients from previous nutrient applications, biological fixation, irrigation or flood water, atmospheric deposition, and soil weathering (Cui et al. 2008; Dobermann et al. 2003a; Witt and Dobermann 2002).
Indigenous nutrient supply determines yield response to fertilisation, where yield response to applied nutrients has been observed to be low when indigenous nutrient supply is high (Kihara et al. 2016; Kihara and Njoroge 2013; Saito et al. 2015, 2019; Vanlauwe et al. 2011). Likewise, use efficiency of fertiliser nutrients is observed to be low at higher soil nutrient supply (Haefele and Wopereis 2005; Haefele et al. 2003; Kihara and Njoroge 2013; Vanlauwe et al. 2011). Fertiliser application rate and timing, weeding time and the associated weed infestation levels, other crop management practices, and climatic conditions also influence fertiliser nutrient use efficiency (Davies et al. 2020; Tippe et al. 2020; Xu et al. 2016; Zemichael et al. 2017). While indigenous nutrient supply is shown to determine fertiliser nutrient use efficiency, its interaction with crop management practices, for instance timing of weeding—the most important component of management practices (Awio et al. 2022), could further influence use efficiency of fertiliser nutrients. However, there is no clear evidence of the effect of interaction between indigenous nutrient supply and crop management practices on fertiliser nutrient use efficiency. Current fertiliser recommendations for site-specific nutrient management are based on indigenous nutrient supply (Chivenge et al. 2021; Dobermann et al. 2002) without consideration for proper weed management, which may make such recommendations inefficient when fertilisers are applied, and weeding is not done timely. Some of the agronomic indices used to describe nutrient use efficiency include agronomic efficiency (AE)—kg grain yield increase kg−1 nutrient applied; recovery efficiency (RE)—kg increase in nutrient uptake kg−1 nutrient applied; and physiological efficiency (PE)—kg grain kg−1 nutrient uptake (Cassman et al. 1998; Dobermann 2007; Li et al. 2014).
In SSA, fertilisers remain an expensive crop production input for most rice farmers, resulting in farmers’ actual application rates being lower than recommended rates. Ensuring good nutrient use efficiency of the applied fertiliser is important for increasing both fertiliser use and rice yield (Tsujimoto et al. 2019; Vanlauwe et al. 2011). This could ensure that farmers achieve positive returns from fertiliser investments, while avoiding negative environmental effects, including contribution to greenhouse gas emissions, and loss of excess nutrients to surface and underground water. The objective of the present study was, therefore, to understand the effect of the interaction between indigenous nutrient supply and management practices in sha** nutrient uptake and use efficiency on-farm, under irrigated lowland rice conditions. Previous studies indicate that an increase in indigenous nutrient supply reduces agronomic and recovery efficiency of applied nutrients, and physiological efficiency of nutrients taken up. The latter implies nutrient concentrations in biomass increase. We hypothesise an interaction between indigenous nutrient supply with timing of weeding and of fertilisation, which are a challenge to rice farmers in Uganda in particular, and SSA at large. Agronomic and recovery efficiency of applied nutrients, and the physiological efficiency of nutrients taken up are expected to decline as indigenous nutrient supply increases, due to a reduction in uptake of applied nutrients and an increase in biomass nutrient concentrations. The reduction in agronomic and recovery efficiencies will be stronger with delayed weeding and fertilisation, while delayed weeding and fertilisation will also reduce the physiological efficiency due to a reduction in uptake of applied nutrients.
Materials and methods
Trial site, design and treatments
The trials used for the current analysis were participatory trials involving farmers and researchers, conducted on farmers’ fields between January and December 2019, in the Doho rice irrigation scheme in Butaleja district (34°02’ E, 0°56’ N), Eastern Uganda. The Doho rice irrigation scheme is the largest public rice irrigation scheme in Uganda (Wanyama et al. 2017), covering an area of 1000 ha, of which 952 ha is cultivated by over 4000 smallholder farmers. The scheme lies at an elevation of 1100 m above sea level and the annual mean temperature in the area is 22.7 °C, ranging from 15.4 to 30.7 °C. It is characterised by a bimodal rainfall pattern, with peaks in March–May and August–October, with a mean annual rainfall of 1186 mm. The soils are Plinthosols, reddish brown in colour, sandy loam, and loam textured (Awio et al. 2022). Currently, the scheme is divided into 11 blocks; each block is sub-divided into 5–15 strips, and each strip has 20–30 farmers.
The scheme has been under lowland rice growing by smallholder farmers for about four decades (Sserunkuuma et al. 2003), where rice is cultivated in a monoculture system, with 2–3 crops planted per year in the same field. Irrigation management is similar across fields based on water release schedules for the different blocks drawn by the scheme’s management, in addition to rainfall. Farmers incorporate rice straw from the previous crop during land preparation; no external sources of organic input are applied. Varied amounts of inorganic fertilisers, especially N, are used by farmers, ranging from 0 to about 50 kg N ha−1 and almost no P and K (Awio et al. 2022). Farmers from all blocks were invited to participate in the trials. The fields used for the participatory trials were from farmers that decided to participate, while participation from all blocks was guaranteed. For each planting period, interested farmers who had fields ready for planting offered their plots for use in conducting the trials. A total of 47 farmers’ fields spread across all the 11 blocks within the scheme were used.
In the trials, farmers differed in nutrient application rates, and on plots with researcher proposed rates, 100, 50 and 50 kg ha−1 N, P and K, were applied, respectively. Treatments included: (1) recommended agronomic practices without fertilisation (RAP) as a control, which represented optimal management practices (Awio et al. 2022). This treatment was jointly managed by farmers and researchers, whereby individual farmers executed a given management practice under the researcher’s supervision (Table 1). (2) Farmers’ practice (FP), which represented all the management practices currently undertaken by farmers. The treatment was fully under farmers’ management, where all farmers implemented their individual management practices, and records of such management practices were taken. (3) Recommended agronomic practices with NPK fertilisation (RAP + NPK), where in addition to optimal management practices, N, P, and K were applied at 100, 50 and 50 kg ha−1, respectively, as urea, triple superphosphate, and muriate of potash. All P was applied as basal, 2 weeks after transplanting (WAT). N and K were split into 50, 25 and 25%, and applied 2 WAT, at panicle initiation and at flowering, respectively. This plot was also jointly managed by the farmers and researchers. (4) Farmers’ best selected management practices as their next feasible step to intensification (farmers’ intensification practice, FIP). Here individual farmers implemented what they considered as their best set of practices to improve their own yield. Hence, different farmers implemented different practices (Table 1). This plot was fully managed by the farmers and records of all management practices were taken.
For all treatment plots, ploughing was done by the farmers following their common practice: two ploughings using either a hand hoe or an ox-plough. First and second ploughing were done at 2–3 weeks and 1 or 2 days before transplanting, respectively. All fields were properly bunded by default because the bunds act as boundaries between farmers’ fields. For practical reasons, levelling was completed in the whole farmer’s field, using a hand hoe, before treatment plots were installed, making all treatments conducted on equally well levelled fields. In addition to rainfall, water was supplied three days per week by irrigation to all treatment plots in a field, based on the water release schedule for the different blocks. For FP and FIP plots, all the farmers applied the fertiliser once, with application timing varying from farmer to farmer. All treatment plots were weeded manually using a hand hoe, once during the crop growth cycle, as is common practice at the study site, with timing of weeding varying among farmers. Bird control was by physical scaring of birds from the grain-filling stage until harvest.
Different farmers implemented different number of treatments, with all farmers having the recommended agronomic practices (RAP) as control plot. An individual farmer’s field was divided into plots and these plots were randomly allocated to the treatments selected and implemented by the farmer, and each farmer was considered a replicate. Planting was done in January, March, April, and August 2019, with overall three crops evaluated across the year. The January crop was the first crop, planted in the dry season, and depended mostly on irrigation water with little rainfall. March and April, and August plantings were the second and third crops, planted in the first and second rainy seasons of the year, respectively, and fully utilised the rainfall with supplementary irrigation. Short-duration rice varieties K 98 and K 85, commonly cultivated by farmers within the study site, were used. Within each farmer’s field, each treatment had a plot size of 10 m × 10 m, and a harvest area of 4 m × 4 m marked from the centre of each treatment plot was used for grain yield assessment.
Estimating effects of indigenous nutrient supply and weeding time delay
To estimate the effect of the indigenous supply of N, P and K of individual farmers’ fields, an estimate of such supply under RAP is needed. Two methods have been used in the literature, one is the amount of N, P or K taken up by a crop species under zero fertilisation, but otherwise good management (Haefele et al. 2003). The alternative method is to measure the amount of N, P or K taken up by a crop while other nutrients are made unlimited through the so-called omission plot technique (Dobermann et al. 2003b; Haefele & Wopereis 2005; Witt & Dobermann 2002). The latter is a better estimate to establish recommendations for optimal fertilisation or a potential supply capacity. The former, though, provides a better estimate of indigenous supply under (good) farmer practice and conditions. As the current paper aims to analyse how the apparent nutrient uptake from fertilisation is affected by the indigenous supply when no nutrients are provided, the uptake under no fertilisation suits best and is thus used as an estimate for indigenous nutrient supply (for N, P and K denominated, respectively, as INS, IPS and IKS).
Weeding time for this paper is defined as the time of weeding (in days) after transplanting. Recommended weeding time was considered as weeding time in RAP plots after transplanting, which was between 14 and 25 days after transplanting. Weeding time delay for a given FP or FIP plot was taken as the number of days weeding was done in FP or FIP plot after weeding in RAP plot, i.e., the difference in weeding time between RAP and FP or FIP plots. Weeding time delay was 0 if weeding in RAP and FP/FIP plots was done on the same day, and greater than 0 if weeding in the FP/FIP plot was done after weeding in the RAP plot. Weeding time delay varied between 0–20 days.
Plant analysis
At harvest, 12 sample hills were systematically selected from within 1 m outside the 4 m × 4 m harvest area (Awio et al. 2022), and plants were cut at soil surface level. Sample hills were threshed, and straw, filled, and empty grains, were separately oven-dried at 70 °C to a constant weight. Dry matter harvest index (HI) was calculated as the ratio of filled grains over total above-ground biomass of the sample hills. For each treatment plot, a sub-sample of the straw, filled, and empty grains was reconstituted to make a single sample. Each sample was ground into a fine powder using a high-speed vibrating sample mill (Model CT 293 Cyclotec™, Foss Analytical A/S, Denmark) for analysis of N, P, and K tissue concentrations. Total tissue N concentration was analysed by the Dumas combustion method using a TruMac® CN (LECO Corporation, USA) elemental analyser. Total P and K tissue concentrations were measured using Inductively Coupled Plasma Mass Spectrometry (ICP-MS) with a Thermo-Fisher Scientific iCAP-Q (Thermo Fisher Scientific, Germany) analyser. Chemical analyses were done at the School of Biosciences laboratory, University of Nottingham, UK.
Plant N, P and K uptake was calculated from concentrations in total above-ground dry matter. Total above-ground dry matter was derived from HI of the 12 sample hills and grain yield (from the 4 m × 4 m harvest area) at 14% moisture content. Indigenous N, P and K supply of each farmer’s field was estimated by measuring N, P and K uptake in total above-ground dry matter of recommended agronomic practices (RAP) plots where no nutrients were applied but otherwise management was optimal. Apparent recovery of N, P and K from applied fertiliser was calculated as the difference between total uptake in the fertilised plot and uptake in the unfertilised plot under RAP.
Statistics
Data were subjected to analysis of variance (ANOVA) using an unbalanced treatment structure in Genstat (19th edition) at 5% probability, where the different farmers’ fields were taken as blocks. Where differences among treatments were significant, Fisher’s unprotected least significant difference test was used to separate treatment means. Homogeneity of variances and normality of data distribution were checked using Bartlett’s and Shapiro–Wilk tests, respectively. During analysis, planting period was included as a covariate; however, its effect alone or in interaction with the different treatments was not significant (p > 0.18) so it was then dropped. To quantify the effect of soil indigenous nutrient supply and management practices on apparent recovery, agronomic and recovery efficiency of applied nutrients, and physiological efficiency of nutrients taken up, all treatment data on indigenous N, P, and K supply, weeding time, fertiliser amount and application time were subjected to multiple regression analysis using a generalised linear model following a stepwise regression procedure.
Results
Agronomic and recovery efficiencies of applied nutrients
Agronomic efficiency (AE) and recovery efficiency (RE) of N, P and K were not significantly different among treatments (p ≥ 0.06, Table 2). However, apparent recovery of fertiliser nutrients differed significantly (p < 0.001) among treatments. AE of N varied from –100 to 68.8, –85.9 to 75.7 and –0.5 to 22.2 kg grain kg−1 N applied, under FP, FIP and RAP + NPK, respectively. RE of N ranged between –2.2 and 1.8, − 1.8 and 2.1, and –0.04 and 0.9 kg N in plant tissue per kg N applied, respectively, under FP, FIP and RAP + NPK. N, P and K apparent recoveries were higher in the RAP + NPK treatment, averaging 43.1, 7.5 and 55.8 kg N, P and K ha−1, respectively. Average N apparent recovery was –2.2 kg ha−1 under FP, which was not statistically different from 3.5 kg ha−1 in FIP plots. P and K apparent recoveries were on average 0.03 and 0.98 kg ha−1, respectively, under FIP.
AE of P or K ranged from –18.1 to 62.0 and –1.1 to 44.4 kg grains kg−1 nutrient applied, respectively, under FIP and RAP + NPK. RE of P was between –0.4 and 0.4 and between 0.01 and 0.4, and for K between –2.2 and 5.1 and between –0.3 and 2.9 kg nutrient in plant tissue kg−1 nutrient applied, respectively, under FIP and RAP + NPK. Negative agronomic and recovery efficiency values indicate poor utilisation of the applied nutrients by crops, whereby their uptake and subsequent use in grain production were lower than when no fertiliser was applied but practices were as recommended (RAP).
Nutrient uptake and physiological efficiency
Uptake of N, P and K was significantly (p < 0.001) different among treatments. RAP + NPK had the highest N, P and K uptake, while N, P and K uptake were similar under RAP, FP and FIP (Table 3). There was a large variation in uptake among plots of the same treatment with and without fertilisation, ranging from 52.9 to 176, 12.7–46.4, and 81.9–324 kg ha−1 N, P and K, respectively (Supplementary Fig. 1), indicating differences in indigenous nutrient supply capacity among farmers’ fields. The different planting periods had no significant effect (p > 0.18) on nutrient uptake under the different treatments (Supplementary Table S1). Physiological efficiency (PE) of N, P and K differed significantly (p < 0.05) among treatments, where RAP, FP and FIP had higher PE of N, P and K compared with RAP + NPK (Table 3). N, P and K PE values were on average 18.9, 12.1 and 11.5%, respectively, lower under RAP + NPK compared with RAP, FP and FIP. There was no statistical difference in PE of N, P and K between RAP, FP and FIP. Likewise, across treatments PE varied two- to three-fold among plots with the same treatment, for N, P and K, respectively, from 30.7 to 73.7, 116–305, and 18.9–49.4 kg grains kg−1 uptake (Supplementary Fig. 2). The significantly lower PE of N, P and K observed under RAP + NPK was mainly due to a larger positive effect of RAP + NPK on N, P and K uptake, compared with a smaller positive effect on grain yield, whereby the increase in uptake was on average twice as much as the increase in yield. This increase in N, P and K uptake resulted in a higher total biomass production with a higher nutrient concentration and a lower dry matter HI for RAP + NPK, compared with RAP, FP and FIP. Lower PE of N could be explained by the large increase (16.1%) in N concentration with a decrease (6.3%) in dry matter HI compared with RAP. For P and K PE, dry matter HI and P and K concentrations could explain roughly to a similar extent the decrease in PE under RAP + NPK, with P and K concentrations in biomass increasing by 5.5 and 7.4%, respectively, compared with RAP (Table 3). The lack of significant differences in PE of N, P and K between RAP, FP and FIP could be attributed to the fact that changes in N, P and K uptake under FP and FIP compared with RAP were roughly the same, while also the magnitude of change in dry matter HI, and N, P and K tissue concentrations were comparable.
Factors driving on-farm use efficiency of applied nutrients
Agronomic efficiency of applied nutrients
Multiple linear regression analyses were made, first with the combined data from plots under farmers’ management practices (combining farmers’ practice and farmers’ intensification practice plots). Agronomic efficiency (AE) of applied N was influenced by soil indigenous N supply (INS), and an interaction between INS and the delay in weeding, compared with recommended weeding time. Indigenous N supply had a negative effect on AE, whereby one kg ha−1 increase in indigenous N uptake resulted in a reduction of AE by 0.8 kg grain kg−1 N applied. The interaction of weeding time delay and INS reduced AE by 0.02 kg kg−1 (Model 1A, Table 4). Agronomic efficiency predictions from the model indicated that an increase in INS with an increase in delayed weeding would result in a reduction in AE, with a larger reduction at higher levels of INS (Fig. 1A).
Agronomic efficiency (AE) of N under FP and FIP (A) and across FP, FIP and RAP + NPK (B) as affected by indigenous N supply (INS; kg ha−1), and the interaction between INS and weeding time delay (days after weeding in recommended agronomic practices, RAP, plot), Doho, 2019. Regression lines are fitted for predicted AE values from Models 1A and 1B, respectively. Data points are observed AE values from FP and FIP; and FP, FIP and RAP + NPK plots, respectively. FP = farmers’ practice; FIP = farmers’ intensification practice; and RAP + NPK = recommended agronomic practices combined with 100, 50 and 50 kg ha−1, respectively, N, P and K application
A second multiple linear regression was made on data combining plots under farmers’ practice (FP), farmers’ intensification practice (FIP) and recommended agronomic practices plus an application of 100 kg N and 50 kg P and K ha−1 (RAP + NPK). Likewise, INS, and an interaction between INS and the delay in weeding compared with recommended weeding time were the main determinants of N AE. In contrast, the AEs of P and K were unaffected by any of these factors. An increase in INS by a kg ha−1 reduced AE of N by 0.7 kg kg−1 (Model 1B). The interaction between INS and weeding time delay reduced AE by 0.02 kg kg−1. AE predictions from the model showed that AE would be reduced with increasing INS and delayed weeding, with larger reductions at higher levels of INS and delayed weeding (Fig. 1B).
Apparent recovery and recovery efficiency of applied nutrients
Multiple linear regression analysis of the combined data from plots under farmers’ management practices (farmers’ practice and farmers’ intensification practice plots) showed that apparent N recovery from applied fertiliser was influenced by soil INS, and an interaction between fertilisation timing and the delay in weeding, compared with recommended weeding time. Apparent N recovery by the rice crop decreased with increasing soil INS, indicating a negative effect of high indigenous N supply on the apparent recovery of applied N (Model 2A, Table 4). Both a delay in weeding time and late fertilisation had a negative effect on apparent recovery of applied N and these effects strengthened each other (Model 2A, Table 4); together they affected the intercept with the x-axis of the relation between apparent N recovery and indigenous N supply, where the intercept shifted to the right with timely weeding (Fig. 2A).
Apparent N recovery under FP and FIP (A) and across FP, FIP and RAP + NPK (B) as influenced by indigenous N supply; and the additional interactive effect of fertilisation time (FT) and weeding time delay (WD), and weeding time delay and N rate. Apparent N recovery was calculated as the difference between total uptake in the N fertilised plots and N uptake in unfertilised plots under recommended agronomic practices (RAP). Regression lines are fitted for predicted apparent recovery values from Models 2A and 2B, respectively. Data points are observed apparent recovery data from FP and FIP; and from FP, FIP and RAP + NPK plots, respectively. FT in days after transplanting, WD in days after weeding in RAP plot, ~ means approximately. FP = farmers’ practice, FIP = farmers’ intensification practice and RAP + NPK = recommended agronomic practices combined with NPK application
Combining data from plots under farmers’ practice (FP), farmers’ intensification practice (FIP) and recommended agronomic practices plus NPK application (RAP + NPK), the multiple linear regression showed that application of N shifted the intercept with the y-axis of the relation between apparent N recovery and indigenous N supply upwards, but this recovery was reduced by both delay in weeding and indigenous N supply (Model 2B, Table 4 and Fig. 2B). There was an indication that lower N applications (for instance, up to 35 kg ha−1) under timely weeding and in fields with indigenous N supply of around 60 kg ha−1 would still have decent apparent N recovery. However, a fortnight delay in weeding would bring apparent N recovery to almost zero, while even at timely weeding such N application rate would make no sense at indigenous N supply levels of around 100 kg ha−1 or higher (Fig. 2B).
Recovery efficiency (RE) of applied nitrogen under farmers' management practices (FP and FIP), and across FP, FIP and RAP + NPK was only significantly (p < 0.001) influenced by a delay in weeding time compared with recommended weeding time. A delay in weeding by one day reduced RE of N by 0.04 kg N in plant tissue kg−1 N applied (Model 5, Table 4). There was a large variation in RE across weeding time delays, where a 14-day delay in weeding compared to recommended weeding time had most of the lowest RE values (Supplementary Fig. 3).
Apparent recovery of P across FIP and RAP + NPK plots was affected by P application rate, whereby recovery of applied P increased with amount of P applied (Model 3, Table 4). Estimation from the regression model showed that P application at rates below 15 kg ha−1 in these fields would not be useful as apparent recovery of the applied P would be negative (Fig. 3A). Apparent P recovery was, however, not affected by soil indigenous P supply (IPS), weeding or fertilisation time (Supplementary Fig. 4). Similarly, apparent recovery of fertiliser K was influenced by amount of K applied, in which apparent recovery increased with increasing K application rate (Model 4, Table 4). Like P, K applications below ca. 15 kg ha−1 would make no sense, as the apparent K recovery would be negative (Fig. 3B). Also, apparent K recovery was not affected by indigenous K supply (IKS), weeding or fertilisation time (Supplementary Fig. 5). Recovery efficiencies of applied P and K were not affected by any of the management factors.
Apparent recovery of fertiliser P (A) and K (B) across FIP and RAP + NPK as influenced by P and K rate, respectively, on-farm, Doho, 2019. Apparent recovery was calculated as the difference between total uptake in fertilised plots and uptake in unfertilised plots under recommended agronomic practices (RAP). The regression line is fitted for predicted apparent recovery values from Models 3 and 4, respectively. Data points are observed apparent recovery values from FIP and RAP + NPK plots. FIP = farmers’ intensification practice and RAP + NPK = recommended agronomic practices plus NPK application
Physiological efficiency of nutrients taken up
Multiple linear regression of the combined data from plots under farmers’ management practices across FP and FIP showed that physiological efficiency (PE) of N was significantly (p = 0.04) influenced by INS, where PE decreased by 0.1 kg grain kg−1 N uptake (kg kg−1) for every kg ha−1 increase in N uptake from the soil (Model 6A, Table 4). Across FP, FIP and RAP + NPK, weeding time delay, and interaction between INS and N rate were the drivers of N physiological efficiency. One day delay in weeding increased PE of N by 0.1 kg kg−1. Interaction between INS and amount of N applied reduced PE by 0.001 kg kg−1 for every additional kg ha−1 N uptake from combined soil and fertiliser sources (Model 6B). Estimated PE values from Model 6B showed that increasing N rate with increasing INS reduced PE of N (Fig. 4). Indeed, PE of N on-farm was generally lower at higher N amounts (100 kg ha−1) compared with lower N rates (Fig. 4). This could indicate that the increase in N availability to crops, from N application and soil supply, resulted in more N uptake by crops which was to a greater extent used to produce additional vegetative biomass rather than extra grain. The increase in the PE of N with delayed weeding could be explained by a larger negative effect of delayed weeding on N uptake, compared with the effect on grain yield, where reduction in N uptake was 16.3%, compared with 11.1% grain yield reduction (Supplementary Table S2). There was also a positive effect of delayed weeding on dry matter HI, in that HI was 3.3% higher under late weeding compared with timely weeding. This could imply that with delayed weeding the crops adjusted their sink size in relation to the source, since uptake and overall tissue concentration decreased, so the plants became more efficient in utilising the available nutrients taken up to produce more grains.
Physiological efficiency of N across FP, FIP and RAP + NPK as affected by weeding time delay (WD; days after weeding in RAP plot) and interaction between indigenous N supply and N application rate on-farm, Doho, 2019. Regression lines are fitted for predicted PE values from Model 4B. Data points are observed PE values from FP, FIP and RAP + NPK plots. FP = farmers’ practice, FIP = farmers’ intensification practice and RAP + NPK = recommended agronomic practices combined with NPK application
The physiological efficiency of P across FIP and RAP + NPK was influenced by IPS and the interaction of P rate and P fertilisation time. Increase in IPS by one kg ha−1 led to a reduction in PE of P by 3.4 kg kg−1. The interaction between P rate and fertilisation time also reduced PE of P by 0.05 kg kg−1 for every additional kg ha−1 of P applied combined with a day delay in P fertiliser application. Predictions from regression Model 7 (Table 4) indicated a larger drop in PE of P at high P rate, compared with lower rates (Fig. 5A), which could imply luxury uptake of P at high rate which was inefficiently utilised by the crops for grain production. The PE of K was determined by IKS and interaction between K applied and weeding time. Similarly for K, an increase in IKS by one kg ha−1 resulted in a reduction in the PE by 0.1 kg kg−1. Interaction of K rate and weeding time likewise reduced the PE of K by 0.01 kg kg−1 for every kg ha−1 increase in K rate with a day delay in weeding (Model 8, Table 4). Predictions of K PE by Model 8 showed that at lower or higher K rates, PE reduced with delayed weeding, however, with larger reductions at higher K rate (Fig. 5B). This could indicate that at lower K rates, timing of weeding was a major factor affecting PE of K, while the interaction effect of K amount and weeding time on PE was more negative at higher K applications.
Physiological efficiency (PE) of P (A) and K (B) across FIP and RAP + NPK as determined by indigenous supply; and interaction between P rate and P fertilisation time (FT; days after transplanting), and K rate and weeding time (WT; days after transplanting), respectively, on-farm, Doho, 2019. Regression lines are fitted for predicted PE values from Model 7 and 8, respectively. Data points are observed PE values from FIP and RAP + NPK plots. FIP = farmers’ intensification practice and RAP + NPK = recommended agronomic practices plus NPK application
Discussion
We observed a large variation in indigenous N, P and K supply at zero fertilisation among rice farmers’ fields in the Doho rice irrigation scheme, Uganda. N and P varied about 2.5-fold among fields while K varied about 3.6-fold (Supplementary Fig. 1). Earlier studies reported similar variations in indigenous nutrient supply among farmers’ fields. For instance, Haefele et al. (2003) and Haefele and Wopereis (2005) reported large variations in indigenous N, P and K supply among rice fields under similar growing conditions in SSA. Large field-to-field variations in indigenous P supply (IPS) of rice fields have also been reported for soils with various geological backgrounds, as well as in soils from adjacent fields (Nishigaki et al. 2019). Similar variations in indigenous supply among fields were reported in maize (Fofana et al. 2005; Tabi et al. 2008) and millet (Fofana et al. 2008) fields in SSA. Likewise, in Asia, where fertilisers have been used over a long time at higher rates than in SSA, Cassman et al. (1996) observed huge variations in indigenous N supply (INS) among rice fields in the tropics under comparable growing conditions, noting that the variation was not only among fields but also in the same field over time. Comparable variations in INS, IPS and indigenous K supply (IKS) have also been reported among rice (Dobermann et al. 2003b; Xu et al. 2017), maize and wheat fields (Chuan et al. 2013; Cui et al. 2008; Xu et al. 2014). Dobermann et al. (2003b) identified soil type, climate, and crop management practices as the causes of the large variation in indigenous supply. The differences in indigenous supply among farmers’ fields in the studied irrigation scheme could not be explained by climate or soil type, as these did not really vary. The large variation in indigenous nutrient supply observed among farmers’ fields in this study is an indication that past crop management must have differed, and hence field-specific nutrient management is essential for individual farmers in this production system, where application rates could be based on farmers’ insights of indigenous nutrient supply under zero fertilisation. This is generally relevant across SSA (Chivenge et al. 2022).
The findings of reduced agronomic efficiency (AE); apparent recovery, hence recovery efficiency (RE); and physiological efficiency (PE) with increasing soil indigenous nutrient supply (Figs. 1, 2, 4 and 5) confirm previous studies on the relationship between indigenous nutrient supply and use efficiency of applied nutrients. In rice fields of SSA, Haefele et al. (2003) and Haefele and Wopereis (2005) reported reduction in fertiliser N, P and K recovery fractions with the increase in indigenous nutrient supply. Relatedly, lower fertiliser N recovery was observed in maize fields in non-degraded soils with higher INS than in degraded soils with lower INS (Fofana et al. 2005). However, in millet grown in infields and outfields (i.e., fields close to and away from the homestead, respectively), fertiliser N and P recoveries were higher in infields with higher indigenous supply than in outfields (Fofana et al. 2008). Samaké et al. (2005) observed that in very degraded soils in outfields, fertilisation may not enhance nutrient uptake hence recovery, as there are too many limiting factors, including biotic stresses like striga for millet. Agronomic efficiency of N and P was also reported to reduce in fields where indigenous supply of these nutrients was high (Kihara & Njoroge 2013; Vanlauwe et al. 2011). Equally, Xu et al. (2017) reported reduction in AE of N, P and K in Asian rice fields with increasing indigenous N, P and K supply. Comparable observations were also reported in maize and wheat (Chuan et al. 2013; Xu et al. 2014). The reduction in fertiliser nutrient use efficiency observed in our study, when soil indigenous nutrient supplies were high, further underscores the need for site-specific nutrient application based on individual field capacity to supply nutrients from indigenous sources. While nutrient use efficiencies reduced with increasing soil indigenous supply, the current study further showed an interaction between indigenous nutrient supply and timing of weeding in influencing use efficiencies of applied nutrients (Figs. 1, 2, 4 and 5B). For instance, the interaction between indigenous N supply and weeding time delay significantly reduced AE of N (Fig. 1), compared with recommended weeding time. Similarly, delaying weeding affected the intercept of the relation between apparent N recovery and indigenous N supply (Fig. 2), strongly reducing N recovery efficiency. This effect of delayed weeding combined with indigenous nutrient supply on fertiliser nutrient use efficiencies suggests that site-specific nutrient application must be combined with timely weeding as site-specific recommendations may be inefficient when farmers apply fertilisers and do not weed timely. Present recommendation for site-specific nutrient application is based on indigenous soil supply but not on weed management (Chivenge et al. 2021; Dobermann et al. 2002), indicating that site-specific nutrient management approaches for SSA farmers should take into consideration proper crop management practices, especially timely weeding, which is critical for crop response to fertiliser application (Awio et al. 2022). This will ensure that farmers apply the right amounts of fertilisers under appropriate management practices and make economic returns on fertiliser investments through enhanced fertiliser nutrient use efficiency. Also, the possibility of within-field variation as observed by previous studies (for instance Cassman et al. 1996) should be considered to demonstrate the importance of adopting precise and variable nutrient rates combined with good agronomic practices to maximise production within a single field, thereby also saving money for the farmers. This requires that farmers are empowered to make judicious management decisions based on the actual status of their fields.
In this study, indigenous N, P and K supply were estimated by measuring N, P and K uptake in RAP plots where no nutrients were applied but management was optimal with the aim to estimate the role of indigenous nutrient supply of the fields under farmer management in the agronomic efficiency of fertiliser applications. An alternative approach to estimate indigenous nutrient supply is using nutrient omission plots. While the method applied here might under-estimate the indigenous nutrient supply, as also noted by Haefele et al. (2003) using the same approach, the alternative method would potentially lead to an over-estimation of indigenous supply capacity for nutrients that are least deficient in the system. Considering the above reasoning, we opted for the approach that takes the observed uptake of non-fertilised plots as estimates of uptake under farmer conditions. We consider this as the indigenous nutrient supply, but not supply capacity. In fact the trend that at higher indigenous nutrient supply fertiliser use efficiency is reduced was also observed by previous studies that used nutrient omission trials in rice and maize fields in SSA (e.g., Haefele & Wopereis 2005; Vanlauwe et al. 2006) and Asia (Dobermann et al. 2003b). Likewise, indigenous nutrient supply values estimated by our approach are comparable to values from omission trials (Dobermann et al. 2003b; Haefele & Wopereis 2005). From a practical perspective, indigenous supply estimated using our approach is the most appropriate and much more relevant in explaining the problem of fertiliser use efficiency that farmers encounter, than using the indigenous supply capacity estimation from an omission plot.
Delayed weeding reduced the apparent recovery, RE and AE of N, and the PE of K, while PE of N increased with delayed weeding. Touré et al. (2009) observed lower fertiliser N AE in unbunded rice plots, which had higher weed biomass, than in bunded plots. Similarly, fertiliser N AE was reported to be three-fold lower in open rice fields where weed pressure was high, than in bunded fields (Becker & Johnson 2001). While all our fields were bunded, late weeding showed similar impact. The reduction in use efficiency due to delayed weeding could be attributed to competition among rice plants and weeds for nutrients from both soil and fertiliser sources. This indicates that appropriate weeding time is necessary to allow plants to efficiently utilise nutrients from indigenous sources, and from fertilisers when farmers decide to apply. The increase in PE of N with delayed weeding could imply that, with reduced N uptake, crops became more efficient in utilising the available N taken up to produce more grains by adjusting their sink size in relation to the source. Delayed application of the fertiliser reduced the apparent recovery of fertiliser N under farmers’ management practices across farmers’ practice (FP) and farmers’ intensification practice (FIP), and PE of P. This could imply that, under farmers’ current N rates, N application needs to be synchronised with the correct crop stage for optimal uptake, probably N application at maximum tillering or panicle initiation stage in fields where indigenous supply is low or at grain filling stage where indigenous supply is moderate or high; this point was also observed by Becker and Johnson (2001) and Becker et al. (2003).
Low PE of N, P and K, and low dry matter harvest index were observed at high application rates under recommended agronomic practices (Table 3). This could indicate increased uptake by crops, which was used to enhance vegetative biomass production rather than grain yield. This may require that fertiliser nutrients, especially N, be more spread at this high rate to include split application at later crop stage as previous studies indicate that late application can enhance grain filling and grain yield (Banayo et al. 2018; Becker and Johnson 2001; Zhou 2017).
Conclusions
Indigenous nutrient supply at zero fertilisation varied largely among farmers’ fields within the same irrigation scheme. Use efficiency of applied nutrients decreased with increasing indigenous nutrient supply, indicating that site-specific fertiliser recommendation is required based on indigenous nutrient supply levels of individual farmer’s fields. Delayed weeding and nutrient application timing also reduced use efficiency of fertiliser nutrients. Moreover, an interaction between indigenous nutrient supply and timing of weeding was observed. Therefore, at current farmers’ fertiliser application rates, appropriate weeding and indigenous soil supply-based fertilisation strategies appear to be the major management practices that need to be developed with farmers to enable improving the use efficiency of applied nutrients, and hence, grain yield. Further participatory trials with farmers is needed to determine the appropriate minimum nutrient rates farmers can apply and the correct crop stage(s) for application based on indigenous supply levels of the soil.
Data availability
The datasets generated and analysed during the current study are available from the corresponding author on reasonable request.
References
Awio T, Senthilkumar K, Dimkpa CO, Otim-Nape GW, Kempen B, Struik PC, Stomph TJ (2021) Micro-nutrients in East African lowlands: Are they needed to intensify rice production? Field Crops Res 270:108219. https://doi.org/10.1016/j.fcr.2021.108219
Awio T, Senthilkumar K, Dimkpa CO, Otim-Nape GW, Struik PC, Stomph TJ (2022) Yields and yield gaps in lowland rice systems and options to improve smallholder production. Agronomy 12(3):552. https://doi.org/10.3390/agronomy12030552
Bado VB, Djaman K, Valère MC (2018) Managing fertilizer recommendations in rice-based crop** systems challenges and strategic approaches. In: Bationo A, Ngaradoum D, Youl S, Lompo F, Fening JO (eds) Improving the profitability, sustainability and efficiency of nutrients through site specific fertilizer recommendations in west africa agro-ecosystems, vol 1. Springer International Publishing, Cham, pp 25–50
Balasubramanian V, Sie M, Hijmans RJ, Otsuka K (2007) Increasing rice production in sub-saharan Africa challenges and opportunities. In: Sparks DL (ed) Advances in agronomy, vol 1. Academic Press, New York, pp 55–133
Banayo NPMC, Bueno CS, Haefele SM, Desamero NV, Kato Y (2018) Site-specific nutrient management enhances sink size, a major yield constraint in rainfed lowland rice. Field Crops Res 224:76–79. https://doi.org/10.1016/j.fcr.2018.05.006
Becker M, Johnson DE (2001) Improved water control and crop management effects on lowland rice productivity in West Africa. Nutr Cycl Agroecosyst 59(2):119–127. https://doi.org/10.1023/A:1017585328904
Becker M, Johnson DE, Wopereis MCS, Sow A (2003) Rice yield gaps in irrigated systems along an agro-ecological gradient in West Africa. J Plant Nutr Soil Sci 166(1):61–67. https://doi.org/10.1002/jpln.200390013
Cassman KG, Gines GC, Dizon MA, Samson MI, Alcantara JM (1996) Nitrogen-use efficiency in tropical lowland rice systems: contributions from indigenous and applied nitrogen. Field Crops Res 47(1):1–12. https://doi.org/10.1016/0378-4290(95)00101-8
Cassman KG, Peng S, Olk DC, Ladha JK, Reichardt W, Dobermann A, Singh U (1998) Opportunities for increased nitrogen-use efficiency from improved resource management in irrigated rice systems. Field Crops Res 56(1):7–39. https://doi.org/10.1016/S0378-4290(97)00140-8
Chivenge P, Saito K, Bunquin MA, Sharma S, Dobermann A (2021) Co-benefits of nutrient management tailored to smallholder agriculture. Global Food Secur 30:100570. https://doi.org/10.1016/j.gfs.2021.100570
Chivenge P, Zingore S, Ezui KS, Njoroge S, Bunquin MA, Dobermann A, Saito K (2022) Progress in research on site-specific nutrient management for smallholder farmers in sub-Saharan Africa. Field Crops Res 281:108503. https://doi.org/10.1016/j.fcr.2022.108503
Chuan L, He P, Pampolino MF, Johnston AM, ** J, Xu X, Zhao S, Qiu S, Zhou W (2013) Establishing a scientific basis for fertilizer recommendations for wheat in China: Yield response and agronomic efficiency. Field Crops Res 140:1–8. https://doi.org/10.1016/j.fcr.2012.09.020
Cui Z, Zhang F, Chen X, Miao Y, Li J, Shi L, Xu J, Ye Y, Liu C, Yang Z, Zhang Q (2008) On-farm estimation of indigenous nitrogen supply for site-specific nitrogen management in the North China plain. Nutr Cycl Agroecosyst 81:37–47. https://doi.org/10.1007/s10705-007-9149-8
Davies B, Coulter JA, Pagliari PH (2020) Timing and rate of nitrogen fertilization influence maize yield and nitrogen use efficiency. PLoS ONE 15(5):e0233674. https://doi.org/10.1371/journal.pone.0233674
Dobermann A, Witt C, Dawe D, Abdulrachman S, Gines HC, Nagarajan R, Satawathananont S, Son TT, Tan PS, Wang GH, Chien NV (2002) Site-specific nutrient management for intensive rice crop** systems in Asia. Field Crops Res 74(1):37–66. https://doi.org/10.1016/j.fcr.2008.04.002
Dobermann A, Witt C, Abdulrachman S, Gines HC, Nagarajan R, Son TT, Tan PS, Wang GH, Chien NV, Thoa VTK, Phung CV (2003) Soil fertility and indigenous nutrient supply in irrigated rice domains of Asia. Agron J 95(4):913–923. https://doi.org/10.2134/agronj2003.9130
Dobermann A, Witt C, Abdulrachman S, Gines HC, Nagarajan R, Son TT, Tan PS, Wang GH, Chien NV, Thoa VTK, Phung CV (2003) Estimating indigenous nutrient supplies for site-specific nutrient management in irrigated rice. Agron J 95(4):924–935. https://doi.org/10.2134/agronj2003.9240
Dobermann A (2007) Nutrient use efficiency—measurement and management. International Fertilizer Industry Association, Paris, France, 30
Fofana B, Tamélokpo A, Wopereis MCS, Breman H, Dzotsi K, Carsky RJ (2005) Nitrogen use efficiency by maize as affected by a mucuna short fallow and P application in the coastal savanna of West Africa. Nutr Cycl Agroecosyst 71(3):227–237. https://doi.org/10.1007/s10705-004-5084-0
Fofana B, Wopereis MCS, Bationo A, Breman H, Mando A (2008) Millet nutrient use efficiency as affected by natural soil fertility, mineral fertilizer use and rainfall in the West African Sahel. Nutr Cycl Agroecosyst 81(1):25–36. https://doi.org/10.1007/s10705-007-9146-y
Haefele SM, Wopereis MCS (2005) Spatial variability of indigenous supplies for N, P and K and its impact on fertilizer strategies for irrigated rice in West Africa. Plant Soil 270(1):57–72. https://doi.org/10.1007/s11104-004-1131-5
Haefele SM, Wopereis MCS, Ndiaye MK, Barro SE, Ould Isselmou M (2003) Internal nutrient efficiencies, fertilizer recovery rates and indigenous nutrient supply of irrigated lowland rice in Sahelian West Africa. Field Crops Res 80(1):19–32. https://doi.org/10.1016/S0378-4290(02)00152-1
Kihara J, Njoroge S (2013) Phosphorus agronomic efficiency in maize-based crop** systems: a focus on western Kenya. Field Crops Res 150:1–8. https://doi.org/10.1016/j.fcr.2013.05.025
Kihara J, Huising J, Nziguheba G, Waswa BS, Njoroge S, Kabambe V, Iwuafor E, Kibunja C, Esilaba AO, Coulibaly A (2016) Maize response to macronutrients and potential for profitability in sub-Saharan Africa. Nutr Cycl Agroecosyst 105:171–181. https://doi.org/10.1007/s10705-015-9717-2
Kwesiga J, Grotelüschen K, Neuhoff D, Senthilkumar K, Döring TF, Becker M (2019) Site and management effects on grain yield and yield variability of rainfed lowland rice in the Kilombero floodplain of Tanzania. Agronomy. https://doi.org/10.3390/agronomy9100632
Li M, Zhang H, Yang X, Ge M, Ma Q, Wei H, Dai Q, Huo Z, Xu K, Luo D (2014) Accumulation and utilization of nitrogen, phosphorus and potassium of irrigated rice cultivars with high productivities and high N use efficiencies. Field Crops Res 161:55–63. https://doi.org/10.1016/j.fcr.2014.02.007
Niang A, Becker M, Ewert F, Dieng I, Gaiser T, Tanaka A, Senthilkumar K, Rodenburg J, Johnson JM, Akakpo C, Segda Z (2017) Variability and determinants of yields in rice production systems of West Africa. Field Crops Res 207:1–12. https://doi.org/10.1016/j.fcr.2017.02.014
Nishigaki T, Tsujimoto Y, Rinasoa S, Rakotoson T, Andriamananjara A, Razafimbelo T (2019) Phosphorus uptake of rice plants is affected by phosphorus forms and physicochemical properties of tropical weathered soils. Plant Soil 435(1–2):27–38. https://doi.org/10.1007/s11104-018-3869-1
Saito K, Diack S, Dieng I, N’Diaye MK (2015) On-farm testing of a nutrient management decision-support tool for rice in the Senegal River valley. Comput Electron Agric 116:36–44. https://doi.org/10.1016/j.compag.2015.06.008
Saito K, Vandamme E, Johnson JM, Tanaka A, Senthilkumar K, Dieng I, Akakpo C, Gbaguidi F, Segda Z, Bassoro I, Lamare D (2019) Yield-limiting macronutrients for rice in sub-Saharan Africa. Geoderma 338:546–554. https://doi.org/10.1016/j.geoderma.2018.11.036
Samaké O, Smaling EMA, Kropff MJ, Stomph TJ, Kodio A (2005) Effects of cultivation practices on spatial variation of soil fertility and millet yields in the Sahel of Mali. Agr Ecosyst Environ 109(3):335–345. https://doi.org/10.1016/j.agee.2005.02.024
Seck PA, Ali AT, Jeanne YC, Aliou D, Wopereis MCS (2013) Africa’s rice economy before and after the 2008 rice crisis. In: Wopereis MCS, Johnson DE, Ahmadi N, Tollens E, Jalloh A (eds) Realizing Africa’s rice promise. CABI, Wallingford, p 436
Sserunkuuma D, Ochom N, Ainembabazi H (2003) Collective Action in canal irrigation systems management: the case of Doho rice scheme in Uganda. Department of Agricultural Economics and Agribusiness, Makerere University, p 22
Tabi FO, Diels J, Ogunkunle AO, Iwuafor ENO, Vanlauwe B, Sanginga N (2008) Potential nutrient supply, nutrient utilization efficiencies, fertilizer recovery rates and maize yield in northern Nigeria. Nutr Cycl Agroecosyst 80(2):161–172. https://doi.org/10.1007/s10705-007-9129-z
Tanaka A, Diagne M, Saito K (2015) Causes of yield stagnation in irrigated lowland rice systems in the Senegal River Valley: application of dichotomous decision tree analysis. Field Crops Res 176:99–107. https://doi.org/10.1016/j.fcr.2015.02.020
Tanaka A, Johnson JM, Senthilkumar K, Akakpo C, Segda Z, Yameogo LP, Bassoro I, Lamare DM, Allarangaye MD, Gbakatchetche H, Bayuh BA (2017) On-farm rice yield and its association with biophysical factors in sub-Saharan Africa. Eur J Agron 85:1–11. https://doi.org/10.1016/j.eja.2016.12.010
Tippe DE, Bastiaans L, van Ast A, Dieng I, Cissoko M, Kayeke J, Makokha DW, Rodenburg J (2020) Fertilisers differentially affect facultative and obligate parasitic weeds of rice and only occasionally improve yields in infested fields. Field Crops Res 254:107845. https://doi.org/10.1016/j.fcr.2020.107845
Touré A, Becker M, Johnson DE, Koné B, Kossou DK, Kiepe P (2009) Response of lowland rice to agronomic management under different hydrological regimes in an inland valley of Ivory Coast. Field Crops Res 114(2):304–310. https://doi.org/10.1016/j.fcr.2009.08.015
Tsujimoto Y, Rakotoson T, Tanaka A, Saito K (2019) Challenges and opportunities for improving N use efficiency for rice production in sub-Saharan Africa. Plant Production Science 22(4):413–427. https://doi.org/10.1080/1343943X.2019.1617638
Vanlauwe B, Tittonell P, Mukalama J (2006) Within-farm soil fertility gradients affect response of maize to fertiliser application in western Kenya. Nutr Cycl Agroecosyst 76(2):171–182. https://doi.org/10.1007/s10705-005-8314-1
Vanlauwe B, Kihara J, Chivenge P, Pypers P, Coe R, Six J (2011) Agronomic use efficiency of N fertilizer in maize-based systems in sub-Saharan Africa within the context of integrated soil fertility management. Plant Soil 339(1):35–50. https://doi.org/10.1007/s11104-010-0462-7
Wanyama J, Ssegane H, Kisekka I, Komakech AJ, Banadda N, Zziwa A, Lubwama KF (2017) Irrigation development in Uganda: constraints, lessons learned, and future perspectives. J Irrig Drain Eng 143(5):10
Witt C, Dobermann A (2002) A site-specific nutrient management approach for irrigated, lowland rice in Asia. Better Crops Int 16(1):20–24
Xu X, He P, Pampolino MF, Johnston AM, Qiu S, Zhao S, Chuan L, Zhou W (2014) Fertilizer recommendation for maize in China based on yield response and agronomic efficiency. Field Crops Res 157:27–34. https://doi.org/10.1016/j.fcr.2013.12.013
Xu X, He P, Zhao S, Qiu S, Johnston AM, Zhou W (2016) Quantification of yield gap and nutrient use efficiency of irrigated rice in China. Field Crops Res 186:58–65. https://doi.org/10.1016/j.fcr.2015.11.011
Xu X, He P, Yang F, Ma J, Pampolino MF, Johnston AM, Zhou W (2017) Methodology of fertilizer recommendation based on yield response and agronomic efficiency for rice in China. Field Crops Res 206:33–42. https://doi.org/10.1016/j.fcr.2017.02.011
Zemichael B, Dechassa N, Abay F (2017) Yield and nutrient use efficiency of bread wheat (Triticum Aestivum L.) as influenced by time and rate of nitrogen application in Enderta, Tigray, Northern Ethiopia. Open Agric 2(1):611–624. https://doi.org/10.1515/opag-2017-0065
Zhou W, Tengfei L, Yong C, Hu J, Zhang Q, Ren W (2017) Late nitrogen application enhances spikelet number in indica hybrid rice (Oryza sativa L.). Sci Agricola 74(2):127–133. https://doi.org/10.1590/1678-992X-2016-0094
Acknowledgements
The authors are grateful to the Netherlands Organisation for Scientific Research through Science for Global Development (NWO-WOTRO), and the Netherlands Universities Foundation for International Cooperation (NUFFIC), for funding this study. We thank the field assistants Angella Lowra Ajam and Zebrone Gessa for assisting in data collection. Thanks to the technical manager at the Doho rice irrigation scheme, Wilberforce Sagula, for the good working environment provided that enabled smooth working with farmers. Farmers are acknowledged for enthusiastically participating in conducting the trials.
Funding
Financial support for this study was provided by the Netherlands Organization for Scientific Research through Science for Global Development (NWO-WOTRO, grant number: W08.270.323), and by the Netherlands Universities Foundation for International Cooperation (NUFFIC, grant number: NFP—PhD.17/0019).
Author information
Authors and Affiliations
Contributions
TA, KS, COD, GWO-N and TS: Conceptualisation, Methodology, Validation. TA: Investigation. TA, PCS, and TS: Formal analysis, Writing—Original draft preparation. KS and COD: Writing—Reviewing and editing.
Corresponding authors
Ethics declarations
Conflict of interest
The authors have no competing interests to declare that are relevant to the content of this article.
Additional information
Publisher's Note
Springer Nature remains neutral with regard to jurisdictional claims in published maps and institutional affiliations.
Supplementary Information
Below is the link to the electronic supplementary material.
Rights and permissions
Open Access This article is licensed under a Creative Commons Attribution 4.0 International License, which permits use, sharing, adaptation, distribution and reproduction in any medium or format, as long as you give appropriate credit to the original author(s) and the source, provide a link to the Creative Commons licence, and indicate if changes were made. The images or other third party material in this article are included in the article's Creative Commons licence, unless indicated otherwise in a credit line to the material. If material is not included in the article's Creative Commons licence and your intended use is not permitted by statutory regulation or exceeds the permitted use, you will need to obtain permission directly from the copyright holder. To view a copy of this licence, visit http://creativecommons.org/licenses/by/4.0/.
About this article
Cite this article
Awio, T., Struik, P.C., Senthilkumar, K. et al. Indigenous nutrient supply, weeding and fertilisation strategies influence on-farm N, P and K use efficiency in lowland rice. Nutr Cycl Agroecosyst 126, 163–180 (2023). https://doi.org/10.1007/s10705-023-10275-z
Received:
Accepted:
Published:
Issue Date:
DOI: https://doi.org/10.1007/s10705-023-10275-z